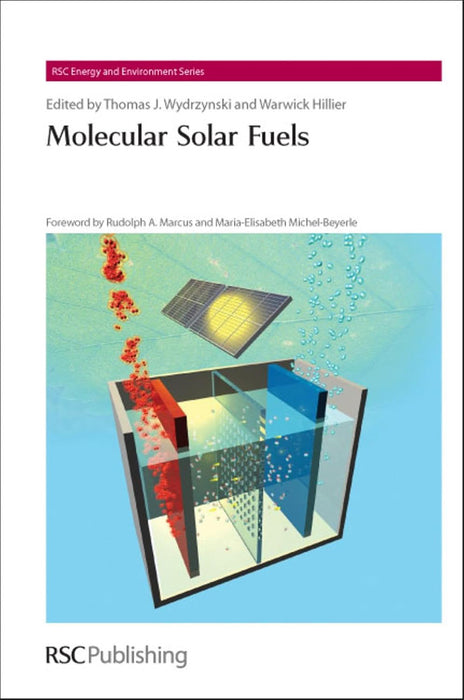
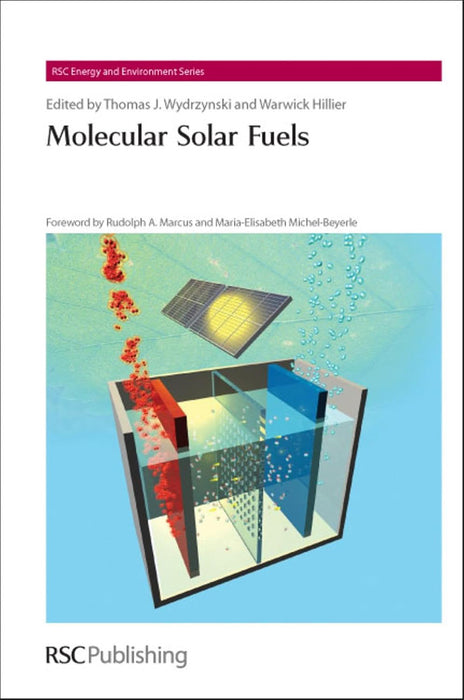
World demand for energy is rapidly increasing and is projected to more than double by the year 2050. Finding sufficient supplies of clean energy for the future is one of the major scientific challenges of today. Sunlight accounts for the largest energy input into the earth's surface, providing more energy in one hour than all of the energy consumed by the entire planet in one year. Over more than 2 billion years, plants, algae and cyanobacteria have evolved the most efficient methods to utilize solar energy, by catalyzing the light-driven splitting of water into molecular oxygen, protons and electrons. If the released protons are captured and reduced to molecular hydrogen by a suitable hyrodrogenase enzyme, then a perfect fuel cycle can be achieved, since the combustion of hydrogen with oxygen produces only water. In the search for clean, energy-rich fuel sources, we can take advantage of the natural photosynthetic and hydrogenase systems by applying and adapting the energy conserving principles that Nature has evolved in these systems and use them to guide the development of synthetic photo- and reductive catalysts for solar energy utilization. The US Department of Energy Basic Sciences Workshops in 2005 on 'Solar Energy Utilization' and in 2007 on 'Catalysis for Energy' identified the development of solar fuels as a key, carbon-neutral, energy resource for the future and hydrogen is one such promising example. The energy released from the combustion of hydrogen with oxygen can be coupled to electrical current generation or the reduction of carbon compounds such as carbon dioxide. If hydrogen could be readily produced from water using solar energy, then an ideal fuel cycle would be possible.
Wydrzynski is a Professor and Head of the Photobioenergetics Group at the ANU (Australian National University) School of Biology where he conducts research on natural and artificial photosynthesis. He is on the Editorial Boards of Photosynthesis Research and Biochimica et Biophysica Acta - Bioenergetics. Professor Wydrzynski received his BA in Biology from the University of Missouri at St. Louis and a PhD in Plant Biology from the University of Illinois at Urbana-Champaign. He subsequently held research posts at the Max Volmer Institute for Biophysical and Physical Chemistry in Berlin, the Standard Oil Company of Indiana (AMOCO Corporation) in Illinois, and the Lawrence Berkeley Laboratory in California. Associate Professor Hillier has authored over forty journal articles, book chapters and conference proceedings. He has also presented his work at a variety of international conferences. Warwick Hillier has a PhD from the ANU and has been a visiting scientist at the National Renewable Energy Laboratory in Golden, USA and a NIH Postdoctoral Research Associate in the Deptartment of Chemistry at Michigan State University. Warwick Hillier is currently an Associate Professor at the ANU (Australian National University) School of Biology. He works on natural and artificial photosynthesis using spectroscopy and molecular biology to study basic problems in electron and proton transfer reactions.
Excerpt. © Reprinted by permission. All rights reserved.
Molecular Solar Fuels
By Thomas J. Wydrzynski, Warwick Hillier
The Royal Society of Chemistry
Copyright © 2012 Royal Society of Chemistry
All rights reserved.
ISBN: 978-1-84973-034-1
Contents
Chapter 1 Harvesting Solar Energy through Natural or Artificial Photosynthesis: Scientific, Social, Political and Economic Implications A. W. D. Larkum, 1,
Chapter 2 Solar Energy Utilisation Ron Pace and Elmars Krausz, 20,
Chapter 3 Converting Photons to Electron and Proton Shifts from Water for Fuel Production Christian Herrero, Cyrille Costentin and Ally Aukauloo, 39,
Chapter 4 Photosynthetic Light-Harvesting Complexes Aaron M. Collins, Jianzhong Wen and Robert E. Blankenship, 85,
Chapter 5 Structure and Function of Photosynthetic Reaction Centres Joanna Kargul and James Barber, 107,
Chapter 6 Photophysics of Photosynthetic Reaction Centres Thomas Renger, 143,
Chapter 7 Photosynthetic O2 Evolution Johannes Messinger, Takumi Noguchi and Junko Yano, 163,
Chapter 8 Substrate and Product Channels in Photosystem II Felix M. Ho, 208,
Chapter 9 Energy from Photosystem II: Manganese Water Oxidation Catalysts Robin Brimblecombe, G. Charles Dismukes, Gerhard F. Swiegers and Leone Spiccia, 249,
Chapter 10 Molecular Ru and Ir Complexes Capable of Acting as Water Oxidation Catalysts X. Sala, L. Escriche and A. Llobet, 273,
Chapter 11 Structure and Function of Hydrogenase Enzymes Wolfgang Lubitz, Hideaki Ogata, Eduard Reijerse and Yoshiki Higuchi, 288,
Chapter 12 Metal Complex of Hydrogenase Active Sites Joe Dawson, Fabio Ghiotto, Jonathan McMaster and Martin Schröder, 326,
Chapter 13 Theoretical Studies of O–O and H–H Bond Formation in Enzymes Per E. M. Siegbahn, 387,
Chapter 14 Redox Active Protein Maquettes: Multi-functional "Green Enzymes" James Murray, 408,
Chapter 15 Light-activated Bioconjugate Complexes David Hvasanov, Daniel C Goldstein and Pall Thordarson, 426,
Chapter 16 Synthetic Photo-catalytic Proteins – a Model of Photosystem II Brendon Conlan, Warwick Hillier and Tom Wydrzynski, 448,
Chapter 17 Wired Reaction Centers Carolyn E. Lubner, Donald A. Bryant and John H. Golbeck, 464,
Chapter 18 Future Perspectives on Solar Fuels T. A. Faunce, 506,
Subject Index, 529,
CHAPTER 1
Harvesting Solar Energy through Natural or Artificial Photosynthesis: Scientific, Social, Political and Economic Implications
A. W. D. LARKUM
School of Biological Sciences, University of Sydney, NSW 2006, Australia
1.1 Introduction
Humans are facing some difficult choices in the near future. It seems highly probable that greenhouse gas emissions will lead to significant global warming over the next 50 years. This will in turn lead to an increasing constraint on use of carbon-based fuels, especially fossil fuels, for transportation, heating, etc. On the other hand solar energy provides our planet with a plenitude of energy, which at the present time is barely utilised by humans. This is a source of energy that is being heralded as the future supply of energy on our planet. And indeed it is clearly a possible source. On the other hand it is important to grasp that this source is not without its own problems, at a number of levels.
Solar energy can be captured and turned into electrical energy by photovoltaic solar panels or by thermal heating systems, with an efficiency of 10% or more. This would displace areas of land or sea used for other purposes or not used at all. Land designated for this purpose would also compete with attempts to use cyanobacteria, algae and plants, ancient photosynthetic organisms on the Earth's surface, to capture solar energy and convert it into bioenergy in the form of stores of organic carbon, albeit by a mechanism with only 0.01–1% efficiency. Nevertheless, this ancient process of photosynthesis is the major driving force for life on our planet. Fortunately, the land or sea needed by photovoltaic/solar thermal systems would be quite small compared with the overall size of our planet: an area of land the size of Egypt or Colombia and slightly larger than that of France would be needed. Much larger areas would be required (>10 fold) if natural photosynthesis were used in the place of photovoltaic solar panels. Furthermore, the use of land for solar harvesting poses ethical problems, which will be discussed below. For example, the use of cropland to produce biofuels has already raised the price of food around the world, even at the moderate levels now applied; so without proper planning very serious ethical problems could arise. Finally, the use of natural photosynthesis to sequester stores of organic carbon for bioenergy is less attractive than it might seem in terms of a carbon and solar footprints, as discussed below.
Use of marginal land to produce lignocellulose products that could form the basis for biofuel production has been an unrealised goal for many years. How realistic is this goal and what are the alternatives? The use of algae in ponds and photobioreactors has also been proposed over recent years. Such systems have a rather large solar footprint and their carbon footprint is not as attractive as it might appear at first sight. Thus it is important to recognise these limitations and plan accordingly.
Of course, another strategy is to re-engineer natural photosynthesis by completely artificial means (see later chapters in this book). As discussed in this chapter, natural photosynthesis is hampered by the fact that the basic mechanisms have evolved over the last 2.5–3 billion years and the ability for retro-engineering has been very limited – and will probably continue to be so even under selection by humans. Therefore, artificial photosynthesis or some form of hydrogen generation, involving photosynthesis, has future potential, despite being in an early stage of development. Such developments have the attraction that they hold out the promise of both small carbon and solar footprints.
1.2 Solar Energy Input to the Earth, and Current and Future Energy Usage by Society
The amount of energy reaching the surface of the Earth has been known for many years and, despite refinement to show decadal variation, the values have remained much the same. The annual value for the amount of energy reaching the Earth's surface is ~2 500 000 ExaJoules (EJ, where EJ = 5 × 1020 Joules yr-1). Discarding the near infra-red wavelengths that are not available to photosynthetic pigments this leaves ~2 200 000 EJ of the available energy per year.
Values for the amount of solar energy at many points on the Earth's surface are available for many sites (e.g., ref. 10). It is also now possible to make good models for any point at the Earth's surface at any time and to compute daily, seasonal, and annual irradiation levels, both for a horizontal surface and for a surface perpendicular to the sun's rays. Perhaps surprisingly, on a cloudless day, the temperate zones offer good levels of solar energy, even though the season is shorter (Table 1.1).
World energy use is approximately 500 EJ per year (based on values for 2008). Of this total, 80–90% is derived from fossil fuels. In equivalent units, this is an average consumption of 16 TeraWatts (1.6 × 1013 W). In comparison with 1980, this represented an approximate doubling of energy use over the previous 25 years. Further, it is calculated to double again by 2040, with China and India accounting for the greatest increases.
On the basis of the Earth's surface area of ~510 000 00 km2 and the value of annual solar radiation (above) one can calculate that currently the world's energy needs would be satisfied with an area of 1 150 925 km2 if 10% of the solar energy could be converted to useable energy, i.e. ~0.02% of the total surface area or 0.07% of the land surface, i.e. equivalent to a country the size of Ethiopia, Egypt or Colombia.
Assuming that natural photosynthesis can convert solar energy at 1% efficiency (a value in the upper range of current plant productivities – see Section 1.5, Figure 1.2 and Table 1.3) the area would be 10 fold the previously mentioned land area, i.e. 11 509 250 km2, or an area a little bigger than Canada and somewhat smaller than the largest country in the world, Russia.
These then are the realistic figures that we need to work with in considering solar energy conversion into energy or organic products by solar panels or by cyanobacteria, algae and plants and/or by artificial photosynthetic systems.
1.3 Photosynthesis on the Earth is an Ancient Process
Solar energy has sustained life by photosynthesis on the Earth from soon after the emergence of the earliest organisms, which appeared about 3.9 Ga (billion years ago). The earliest form of photosynthesis, which may have evolved 3.5 Ga, occurred in an environment of low atmospheric oxygen, high levels of reducing molecules, such as methane, H2S (and other sulphur compounds) and H2 gas, which were used as the oxidising agents necessary for photosynthesis. However, as the reducing atmosphere became exhausted by (i) the activities of the earliest organisms and (ii) the out-gassing to space of gaseous hydrogen, the need for using water as a source of hydrogen placed a strong selection pressure on the evolution of photosynthesis where oxidation occurred by extracting hydrogen from water. This occurred by the evolution of the water splitting mechanism of oxygenic photosynthesis – so called because in the process of using the hydrogen from water, oxygen is released as a byproduct. The first organisms to perform this revolutionary process were undoubtedly cyano-bacteria (or proto-cyanobacteria), which were derived from primitive anoxygenic photosynthetic bacteria.
It is oxygenic photosynthesis, performed by cyanobacteria and their eukaryotic successors, algae and land plants, which is the major process of solar energy conversion on the Earth today (Figures 1.1 and 1.2). And this is also by far the most important process for the input of organic energy into the biosphere: chemical, thermal and radioactive inputs being by comparison negligible.
Since the evolution of the basic mechanisms of photosynthesis took place over 2 billion years ago it is perhaps not surprising that cyanobacteria, algae and land plants all employ a rather uniform chemical apparatus to transform solar energy into the energy stored in organic molecules: the basic mechanisms must have evolved rapidly with the early evolution of cyanobacteria, perhaps 2.7 to 2.4 Ga. Once cyanobacteria came into symbiotic association with eukaryotes (in algae and land plants) it seems that the opportunities for evolution of the basic mechanisms were very restricted. As a result it is not difficult to compute the efficiencies of this rather uniform process in all the different organisms that use oxygenic photosynthesis; the presence of a wide variety of photosynthetically-active pigments in algae make this already diverse group of protists even more diverse in terms of light-harvesting strategies, but nevertheless their absorption properties can be computed quite simply.
The basic equation of photosynthesis is
CO2 + H2O + energy -> [CH2O] + O2
where [CH2O] represents a portion of an organic molecule. This equation allows us to compute the organic production of oxygenic photosynthetic organisms and, from a knowledge of the input of solar energy to our planet, to then calculate realistic rates of solar energy conversion into organic molecules (Table 1.2).
While the basic steps in photosynthesis can be quite efficient, ranging from 99% efficiency for many light-harvesting processes to 70–80% for bacterial reaction centres and photosystem I of oxygenic photosynthesis, this is not the case for the overall process of oxygenic photosynthesis. Losses in efficiency in the primary processes, i.e., up to the stable conversion of CO2 into organic matter, occur at a number of levels and are discussed in the next section (Section 1.4, Figure 1.3).
Finally, it is clear that photosynthesis by land plants is more efficient than that of photosynthetic organisms in the sea (cyanobacteria, algae and a few higher plants – mainly seagrasses). This is clearly shown by the fact that the surface of the globe that is covered by land is only about one third of the total, yet the primary production on the land accounts for about half of the total primary production, despite the relative large areas of desert on the land area (Figure 1.1).
1.4 Inefficiencies in Oxygenic Photosynthesis
1.4.1 Losses in Absorption
The major flows of energy through the biosphere are set out in Figure 1.2. In considering losses of light (excitation energy) before it enters the photosynthetic process, one must consider, principally, (i) losses in the medium, (ii) losses at the plant/algal interface and (iii) losses in the photosynthetic absorption process.
For land plants, losses in the medium (air) are negligible (Figure 1.3A), but for aquatic algae they may be significant; and if the algae live at any depth they may be considerable (Figure 1.3B). Thus, in developing schemes for algae the use of shallow ponds with thin layers of algae is the preferable strategy – and this necessarily rules out considerations of utilising the ocean or large lakes.
For losses at the plant/algal interface, there will inevitably be scattering or reflection of light (Figure 1.3A and B). However, what is more important is how the photosynthetic light-harvesting system is arranged. In land plants, a major consideration is the absorption of light through the plant canopy. In aquatic systems it is the arrangement of algal cells (unicellular, usually) in the water column: here the most efficient arrangement would be to form a thin algal film or stirred algal suspension: but this is impossible in almost all natural situations. The problem is the rapid reduction in light as it penetrates the plant canopy or algal suspension, and light-harvesting over a range of light intensities that may range from inhibitory to light-limiting; and the fact that the inability of the organism to match this changing situation quickly results in large inefficiencies. Since light in the sea either encounters a cell with high pigment content or penetrates a rather transparent medium, an inefficiency known as the package effect occurs, and this further lowers absorption effects in the ocean. Furthermore, harm can be done to the photosynthetic apparatus at high light-intensities, and as a result plants, algae and cyanobacteria put into place a system whereby light energy is wasted by being converted to heat at high intensities (a process called down-regulation) and this further reduces efficiency (see below).
Losses due to plant pigmentation will vary depending on the type of photosynthetic organism. For land plants with their fairly uniform pigmentation and lack of efficient pigments in the green region of the spectrum (Green Window), the losses are quite significant. For algae, the extent of losses are more variable; the absorption spectra of typical algae (e.g. ref. 16) reveal how variable this range may be. The losses range, typically, from 50% for a cyanobacterium, with phycoerythrin, to 75% for a green alga. Clearly, a cyanobacterium with phycoerythrin would be the best photosynthetic organism to use in harvesting solar energy: however, there would be hidden costs in the extra energy needed to synthesise the additional light-harvesting machinery and in the extra nitrogen needed for the extra protein demand of the phycoerythrin.
1.4.2 Efficiency of Primary Photosynthetic Energy Conversion
The reaction centres and light-harvesting assemblies of photosynthesis are incredibly efficient compared with man-made engines. At low to medium light input levels the light-harvesting assemblies can pass on resonance energy at above 95% efficiency. Once this energy is delivered to the reaction centre (Figure 1.4), the basic reactions function at high efficiency (>80) at low to medium energy input. At higher energy levels, three processes lead to inefficiency and damage: (i) double turnover events and relaxation of high-energy states, (ii) photodamage due to accumulation of reactive oxygen species (ROS), and (iii) down-regulation of incoming energy by conversion to heat (non-photochemical quenching: NPQ).
These three processes reduce the efficiency of solar energy conversion in the reaction centres (RCs) from ~80% at low to medium light intensities, to well below 30% at the highest intensities (see below). The situation is also made worse by the fact that photodamage, which occurs at high light intensities during the middle hours of the day, is a long-term event and recovery may take several hours, so that even during the afternoon, when light intensities are lower, the efficiency of photosynthesis is significantly impaired (and in cold climates the effect may linger to the next day). Thus, the quantum yield of land plant and microalgal photosynthesis drops from ~80% under ideal (very low light) conditions to <20% under high light conditions where photoinhibition and NPQ occur.
(Continues...)
Excerpted from
Molecular Solar Fuels
by
Thomas J. Wydrzynski, Warwick Hillier
. Copyright © 2012 Royal Society of Chemistry. Excerpted by permission of The Royal Society of Chemistry.
All rights reserved. No part of this excerpt may be reproduced or reprinted without permission in writing from the publisher.
Excerpts are provided by Dial-A-Book Inc. solely for the personal use of visitors to this web site.
Read less
- Publisher: Royal Society of Chemistry
- Publisher Imprint:
- Publication Date:
- Pages: 576
- ISBN13: 9781849730341
- Item Weight: 1050 grams
- Original Price: 144.99 GBP
- Edition: N/A
- Binding: Hardcover